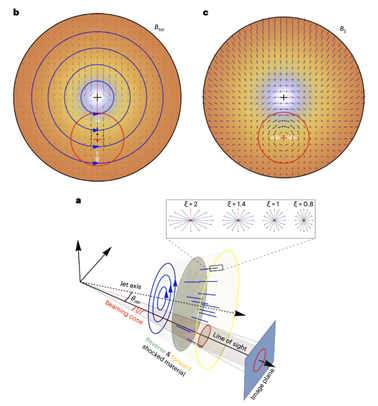
Polarized optical afterglow reveals magnetic field structure in GRB jets and the gamma-rays linked to scattered radiation by reverse shock heated electrons
Gamma-ray bursts (GRBs) are produced by ultra-relativistic jets that are slowed down by their interaction with the surrounding external medium. In the process, two shocks are launched – a forward shock that propagates ahead of the jet and shocks the swept up external medium, and a reverse shock that propagates into the jet, shocks the jet material and slows it down. These shocks accelerate electrons to relativistic energies and also generate in-situ or amplify weak pre-existing magnetic fields. The shock accelerated electrons then lose their energy by producing synchrotron photons that are observed as the broad band (from radio to gamma-rays) and long-lasting (over weeks, months, and years) afterglow emission.
The structure of the shock produced (likely behind the forward shock) and/or amplified (likely in the reverse shock) magnetic field is not clearly known. Some theoretical models and simple particle-in-cell numerical simulations propose that relativistic collisionless shocks in very weakly magnetized external medium produce small (particle skin-depth) scale magnetic fields, via a filamentation/Weible type instability, just behind the shock front and that this field is completely perpendicular to the shock normal (along the direction of the local velocity of the jet material). However, in a realistic GRB jet, as the shocked fluid propagates away from the shock front, the magnetic field is expected to be stretched out along the direction of the shock normal, thereby creating a perpendicular magnetic field component that dominates in large parts of the shocked material. This is expected to occur at the forward shock, but may not always be expected at the reverse shock. Depending on the composition and launching mechanism of the jet, both of which are highly uncertain at this point, it is possible that GRB jets are launched Poynting-flux dominated and contain a large-scale ordered toroidal magnetic field in the plane perpendicular to the jet motion (see schematic figure).
Figure: (a) The two shocked regions behind the forward (yellow) and reverse (green) shocks and the geometry of the magnetic field (blue lines). The forward shocked region contains a small-scale magnetic field stretched in the radial direction and the reverse shocked region contains a large-scale globally ordered toroidal magnetic field axisymmetric around the jet axis. The inset shows the stretching of field lines as the shocked material moves further downstream of the shock. (b) Polarization map (red ticks) and emissivity angular structure (colored gradient) of the reverse shock emission. The observer’s line-of-sight (red plus) is misaligned from the jet axis (blue plus). The observed emission is dominated by that arising from within the beaming cone (red circle), for which the net polarization angle is along the line connecting the jet axis to the line-of-sight. (c) Same as (b) but now showing the polarization map for the radially stretched
magnetic field seen here in projection. The net polarization angle is now perpendicular to that shown in (b).
Fortunately, the linear polarization signature of the afterglow synchrotron emission can be used to learn about the magnetic field structure in the two shocked regions. If the jet contains a large scale ordered magnetic field then the polarization of the reverse shock emission is expected to be much larger (up to few tens of percent) than that from the forward shock (up to only a few percent) as the latter contains small scale magnetic fields that are also axisymmetric around the observer’s line-of-sight. In addition, if the field is indeed stretched along the radial direction behind the forward shock, then the 90 degrees difference in the orientation of the two magnetic fields behind the forward and reverse shocks will likewise produce a 90 degree offset in the polarization angles of the synchrotron emission from the two shocked regions.
This 90 degrees offset in the polarization angle between the reverse and forward shock emissions was revealed for the very first time in a recent work published in the journal Nature Astronomy (https://www.nature.com/articles/s41550-023-02119-1), that involves two ARCO members (Ramandeep Gill and Jonathan Granot) who made important contributions to the theoretical interpretation and modeling of the observations. This inference was made possible by performing optical polarimetry of the afterglow emission of GRB 180720B from the early-time reverse-shock dominated phase (showing rapidly fading emission) to the late-time forward shock-dominated phase (see Figure).
Figure: (a) Optical afterglow lightcurve (red points) of GRB 180720B shown with that of two field stars (blue and yellow). (b) Polarization degree. (c) Polarization angle. The reverse shock emission dominates in the green shaded time interval and the forward shock emission dominates in the yellow shaded interval. The blue shaded interval shows a highly variable polarization evolution that may be attributed to turbulence in the shocked regions.
The optical emission was accompanied by GeV gamma-rays that were detected by the Fermi-LAT instrument in space. Remarkably, the GeV emission showed a lightcurve decay trend very similar to that of the optical when it was dominated by emission from the reverse shock, which indicated that both emissions were most likely coming from the same region of the flow obeying the same dynamical evolution. However, the GeV emission cannot be explained by the extension of the synchrotron component, that produced the optical emission, to higher energies. This is because the reverse shock emission here was produced after the reverse shock had already crossed and shocked all of the jet material, leaving no fresh electrons to be shock accelerated to high energies, thereby completely suppressing any GeV synchrotron emission.
One of the favored mechanisms to produce high energy emission from synchrotron-emitting relativistic electrons is inverse-Compton scattering by the same population of electrons in a process dubbed synchrotron self-Compton (SSC). Time-dependent spectral modeling that accounted for both synchrotron and SSC cooling of electrons in the two shocked regions confirmed the hypothesis, and also clearly demonstrated for the first time, that the GeV afterglow is simply the SSC emission from reverse-shock accelerated electrons (see figure
Figure: Broadband afterglow lightcurve of GRB 180720B and its modeling. Emission from both the reverse shock (dotted lines) and forward shock (dashed lines) is shown, accounting for both synchrotron and SSC cooling of shocked electrons. Both the optical and GeV emission before 1e3 sec is produced by reverse shock accelerated electrons after the shock has crossed the ejecta.
Both observations and their theoretical interpretations have important implications for the composition and launching mechanism of GRB jets as well as particle acceleration and radiative processes at collisionless relativistic shocks.
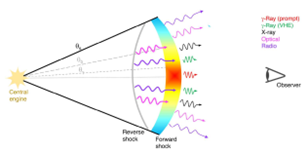
Jet Angular Structure Revealed by the Brightest Gamma-Ray Burst of All Time
The ultrarelativistic (moving at extremely close to the speed of light) jets in Gamma-ray bursts (GRBs) are launched by a rapidly spinning central compact source – a black hole or a magnetar (a highly magnetized, rapidly spinning neutron star). GRBs divide into two classes - short duration GRBs (sGRBs, whose gamma-ray emission lasts less than about two seconds) that are created by the merger of two neutron stars or a neutron star and a black hole, and long duration GRBs (lGRBs, whose gamma-ray emission lasts more than about two seconds) that are produced by the explosion of particularly massive and rapidly spinning stars. It has been known for some time that the angular structure of lGRB jets must be affected by their interaction with the exploding progenitor star’s envelope as the jet bores a hole through it and breaks out. This is well corroborated by advanced numerical simulations.
What is not well known is the exact angular structure these jets possess after they break out.
The jet sweeps up the surrounding medium, driving a strong relativistic shock into it, producing long-lived (up to weeks, months or even years) multi-wavelength (from radio to high-energy gamma rays) afterglow emission by synchrotron radiation in the shocked external medium. This afterglow emission encodes vital information about the jet’s angular structure, which can help unravel it. Most afterglows are well explained as arising from a uniform conical jet with sharp edges. The existence of sharp edges is revealed through a phenomenon called a “jet break” , a steepening of the afterglow lightcurve (an increase in the flux decay rate) when emission from the jet edges reaches us. The sharpness of this break also depends on the radial density profile of the surrounding external medium (which may decrease with distance for the pre-explosion stellar wind of the progenitor massive star).
A puzzling feature that has been seen in some of the bright GRBs is that the jet break is much shallower than what is expected from theoretical models of a sharp-edged conical jet. One explanation for this behavior is that the jet does not have sharp edges, and instead it has smooth extended “wings” where its energy per solid angle very gradually decreases with the angle from the jet’s symmetry axis, dubbed “shallow jets”. Such an angular profile may be described by the jet having a narrow core surrounded by wings where the energy per solid angle and Lorentz factor gradually drop as a power law with the angle from the jet’s symmetry axis.
Thus far, lGRB afterglow observations did not offer any clear evidence to substantiate the presence of any angular structure. This changed on October 10, 2022 when an extremely luminous GRB 221009A was detected by a number of X-ray and gamma-ray detectors in space. Its gamma-ray emission lasted over 600 seconds, and had an isotropic-equivalent gamma-ray energy upwards of 1E55 erg, the largest energy detected for any GRB ever! In addition, it was also relatively close to the Earth (“only” 2.4 billion lightyears), leading to a huge energy fluence, about a hundred times larger than the previous record holder. This garnered the GRB the title of the B.O.A.T (brightest of all time) burst. Not only that, the burst was also very bright in TeV gamma-rays, where the Large High Altitude Air Shower Observatory (LHAASO) on the ground detected more than 64,000 (>0.2)TeV photons, which is unprecedented.
The afterglow of GRB 221009A also showed a shallow achromatic break in the lightcurve, where the achromatic nature is indicative of a geometric, rather than spectral, effect caused by the jet’s angular structure. Several works tried to explain the multi- waveband afterglow lightcurve using canonical models of a uniform conical jet, but they were unable to explain some key features including the shallow break and especially the radio afterglow lightcurve.
Fig. 1: (Left) The angular structure of the jet featuring a narrow uniform core with angular size θγ surrounded by power-law wings in energy with a shallow break at angle θb. Two emission components are realized, with X-rays coming from the shocked matter behind the forward shock and radio emission from the shocked matter behind the reverse shock. (Right) Multiwaveband (Radio, optical, X-ray) model spectral fit from the two emission components to afterglow observations at different times. The dotted curves show the contribution from the reverse shock.
An important revelation that explained these two key features was made in a study published in the journal Science Advances (O’Conner et al. 2023, Sci. Adv., 9, eadi1405) by an international team of researchers, that included three members of the ARCO (Paz Beniamini, Jonathan Granot, and Ramandeep Gill) who made important contributions to the study. This work showed that an ultrarelativistic structured jet with a uniform narrow core surrounded by shallow power-law wings in energy (see left panel of Fig.1), and that is viewed close to the jet core boundary, is the best explanation for the shallow jet break in the afterglow lightcurve. Furthermore, it argued that the X-ray afterglow is entirely powered by emission coming from shocked external matter behind the forward (i.e. afterglow) shock, while the radio afterglow is dominated by emission from the reverse shock that decelerates the original GRB jet material.
The above work featured all of the important ingredients but lacked self-consistency in that the reverse shock region was assumed to be uniform in energy (per solid angle), different from the angular structure assumed for the forward shock region, and the dynamical evolution of the two regions was uncoupled.
Fig. 2: (Left) Multiwaveband model spectral fit to afterglow observations of GRB 221009A. The solid curve shows the total spectrum that constitutes emission from the forward shock (dashed line) and the reverse shock (dotted line). (Middle) Model spectral fit to the radio afterglow shown at different times. (Right) Model lightcurve fit to the afterglow lightcurve, shown for different energies.
This shortcoming in the model was addressed in a follow-up study, now published in MNRAS Letters (Gill & Granot, 2023, MNRAS, 524, L78), by the ARCO members Ramandeep Gill and Jonathan Granot. This work presented a more self-consistent afterglow model that calculated the coupled dynamical evolution of the forward and reverse shocked regions while assuming a common shallow angular structure, in both energy (per solid angle) and bulk Lorentz factor, for both regions. This kind of semi-analytical afterglow model that features a jet with angular structure had never been used for comparison with observations. This study confirmed the jet angular structure assumed in the earlier paper by O’Conner et al. 2023, and definitively showed that the radio afterglow emission is indeed produced by the reverse-shock heated original jet material and even that emission requires the jet to have angular structure.
The unprecedented multi-waveband afterglow observations of the extremely bright GRB 221009A, and the theoretical models put forth in the two works to explain the afterglow, have advanced our understanding of GRB jets. They have shown that many bright GRBs that show a shallow achromatic steepening in their afterglow lightcurves can be explained by the jets having a shallow energy angular structure, which is more realistic and also expected on theoretical grounds and from numerical simulations of powerful GRB jets breaking out of the stellar envelopes of their progenitor stars.
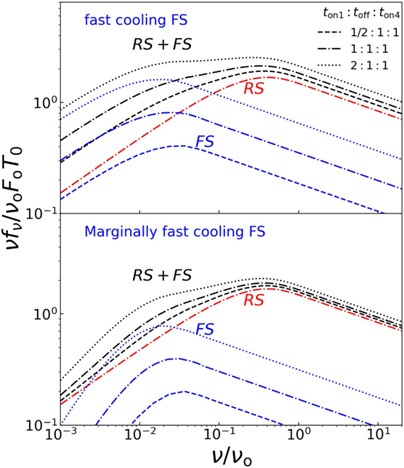
Prompt gamma-ray burst emission from internal shocks – new insights
Link to paper: https://academic.oup.com/mnrasl/article/528/1/L45/7382213
A long standing problem in the literature concerns the shape of the time-integrated spectrum of the prompt phase of GRBs. The spectrum typically shows two spectral features - a high energy peak at a few MeV and a low-energy spectral hump /break at a few hundreds of keV. In this work, we consider an internal shocks scenario where a central engine ejects two cold (faster and slower) shells with constant source power during shell ejection. The faster shell collides with the slower shell at a distance from the central engine. Each such collision produces two shock fronts - a reverse (forward) shock front propagating into a faster (slower) shell. The physical conditions in the two shocked regions are intrinsically different and naturally provide two zones of radiation. The observed radiation is due to contributions from both these shocked regions. We assume synchrotron radiation as the emission mechanism. In our set-up, the reverse shock is stronger, dominates the overall radiative efficiency and gives rise to the MeV peak in the time-integrated spectrum (see Fig. 2). The forward shock contribution can be seen as a low-energy spectral break or a bump. This bump feature has been traditionally interpreted as a "photospheric" feature originating from an optically thick regime. We propose a weaker forward shock in the optically thin regime as an alternative/additional candidate to this interpretation. It must be noted these features arise purely from the shock hydrodynamics alone without tweaking the microphysical parameters in both the shocked regions (taken to be the same in both the regions in our study). And since we naturally have two shocked regions (zones of radiation) our model has a distinct advantage over the one-zone models which require highly-tuned physical conditions.
Figure shows the time-integrated synchrotron spectrum of Prompt Gamma-ray burst for internal shocks as the underlying dissipation mechanism. The contribution from the forward and the reverse shocked region are shown in blue and red curves respectively. The observed spectrum (shown in black curve) is the summation of the contribution from both these regions. The two panels differ in the synchrotron cooling regime for the forward shocked region (marginally fast cooling and fast cooling in the upper and lower panels respectively). The reverse shock is stronger and gives rise to the MeV peak (fast cooling) while the weaker forward shock gives a few hundred keV feature as either spectral bump (marginally fast-cooling) or a spectral break (fast-cooling).
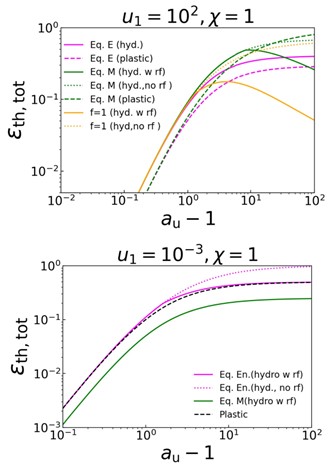
Internal shocks hydrodynamics: the collision of two cold shells in detail
Link to paper: https://academic.oup.com/mnras/article/528/1/160/7492797
Internal shocks are ubiquitous in astrophysical scenarios where outflow has matter moving at variable speeds. The faster trailing material collides with the slower leading material leading to the formation of shocks. These shocks dissipate part of the kinetic energy of the material into internal energy. A fraction of the internal energy is then channeled into electromagnetic radiation for an underlying emission mechanism. Estimation of how efficiently the shocks dissipate energy is a necessary ingredient for application in any specific astrophysical scenarios. However, most works on internal shocks make use of ballistic approximation where plastic collision of two point-like masses is assumed. But realistic estimates of thermal efficiency require solving for the shock hydrodynamics post collision. In each collision two shock fronts are generated with different shock strengths and propagation velocities and internal energy dissipation rates. In this work we undertake such an exercise and find that there can be significant differences between the efficiency estimated from the ballistic approach and the hydrodynamic approach. This is especially important when the relative velocity of the colliding masses are high. At this limit, the ballistic approach suggests very high thermal efficiency. The hydrodynamic approach suggests otherwise. When one treats the hydrodynamics we need to take into account rarefaction waves which can catch up with either of the shock fronts and halt dissipation. The rarefaction waves are triggered effectively at high relative proper speeds and lead to partially shocked shells and hence the rapid drop in thermal efficiency (see Fig 1.) This important consideration needs to be taken in any analysis of an astrophysical scenario.
The figure compares the thermal efficiency from the ballistic (dashed curves) and the hydrodynamic approach (solid curves) as a function of the relative proper speed of the colliding objects. The upper and lower panel is for collision of bodies moving at relativistic and Newtonian speeds respectively. It can be seen that at very low speed contrast the hydrodynamic efficiency is higher than the ballistic efficiency while at very high proper speed contrast the hydrodynamic efficiency is much smaller due to rarefaction waves leading to partially shocked shells.
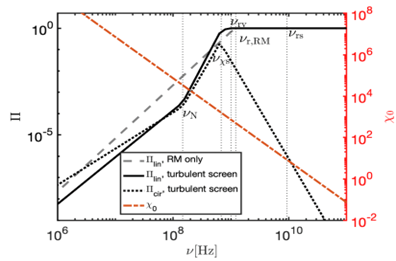
Exploring the environments of FRBs with polarization and Rotation Measure studies
Fast Radio Bursts (FRBs) are brief (typically lasting a few milliseconds), bright flashes of radio waves mostly arriving at Earth from distant galaxies. Some of the sources of FRBs have produced many observed bursts, while the majority have only been detected once so far. In one remarkable case in 2020, an FRB source was discovered in our own Galaxy. The source was identified as a known highly magnetized neutron star (called a ‘magnetar’). This has confirmed the working model, based on various less direct clues, that was held by the majority of astrophysicists studying FRBs at the time. However, the source of the Galactic FRB is much less active than some of the much more distant sources. It therefore remains unclear whether all FRBs arise from magnetars, and if so what conditions might cause a magnetar to produce such signals.
In many bursts, it is possible to measure not only the intensity of the signal but also a property known as its polarization. The very large linear polarization fraction detected in various FRBs suggests that they are produced in strongly magnetized environments (and was one of the early indications towards a magnetar origin). However, other FRBs show a much-reduced fraction of linear polarization or a large fraction of circular polarization. How should these features be understood? Can one emission site and mechanism produce such a large variety of observable features?
As radio waves propagate, they can get deflected and scattered by turbulent and ionized material (plasma) within the interstellar medium. This is a well-known phenomenon that affects radio sources. It leads to a temporal broadening of the radio signal, to modulations in the spectrum (i.e. amount of radiation received per unit frequency) and, under certain condition, it can induce also temporal variability in the observed radiation. In a work from 2022, Dr. Beniamini and collaborators have shown that in addition to the effects mentioned above, the propagation through the plasma (if the medium is sufficiently magnetized), can also affect the observed polarization. It can naturally lead to significant induced circular polarization and/or can cause depolarization of the radiation, both of which are especially pronounced at lower frequencies. This propagation effect could explain the variety of observed behaviors in FRB polarizations, without requiring the emission mechanism to vary hugely from one burst to another. Indeed, the predictions of the model align nicely with observations of various FRBs with well-studied polarization properties. However, these provide only tentative evidence. A smoking gun of such a scenario would be the measurement of a large (and strongly time variable) value of the quantity known as the ‘rotation measure’, which measured the strength of the magnetic field through the column of plasma that the radiation has propagated through
In the meantime, one of the highly active repeating sources of FRB bursts, FRB 20190520B, was monitored in great detail by some of the largest radio telescopes on Earth, for a period of over seventeen months. This monitoring revealed fluctuating polarization properties, with a reduction of polarization at lower frequencies, and a very large rotation measure. Indeed, this rotation measure was fluctuating by a huge amount and has flipped its sign from positive to negative and vice versa during the observation period. These findings matched well the results of the work mentioned above, and in 2023 were published in a paper in Science by Dr. Beniamini and collaborators (see also Open University announcement). In the case of FRB 20190520B, the analysis reveals that the turbulent plasma is likely to be highly magnetized and to reside very close to the FRB source itself. The exact origin of this plasma is still an open question, and it might indicate a magnetized wind from a neutron star surface or an outflow from a binary companion of the object producing the FRB. Either way, this type of analysis presents a new way to constrain the nature of FRB sources and their surroundings and brings us one step closer towards deciphering the mysterious nature of FRBs.
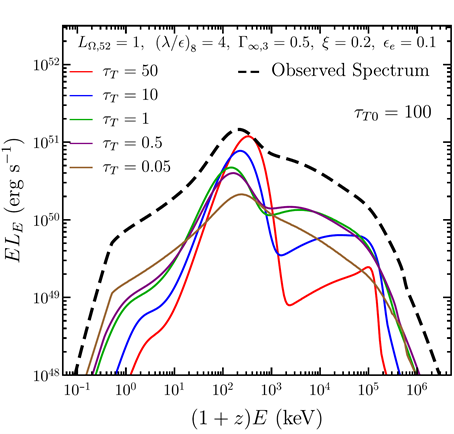
GRB spectrum from gradual dissipation in a magnetized outflow
Gamma-ray bursts (GRBs) are the most (electromagnetically) luminous transient phenomenon in the Universe, outshining the entire gamma-ray sky during their brief prompt emission phase. The latter typically lasts for ~0.03-1 s (in short-hard GRBs, produced by mergers of two neutron stars or a neutron star and a black hole) or ~3-300 s (in long-soft GRBs, produced during the explosion of massive, rapidly rotating stars, accompanied by a powerful supernova explosion). This emission is powered by a relativistic jet but the exact radiation mechanism is still a mystery. Most popular explanations include synchrotron emission from relativistic electrons with a power-law energy distribution, or Compton scattering of thermal photons trapped in the jet by warm electrons. Both of these emission mechanisms often feature in a general class of photospheric emission models that include a prominent quasi-thermal spectral component.
In this work, Dr. Ramandeep Gill, Prof. Jonathan Granot and Dr. Paz Beniamini (all from ARCO) studied how the non-thermal smoothly broken power law spectrum of the prompt GRB emission is produced in magnetized jets. Such jets are accelerated by the gradual and continuous dissipation of the magnetic field energy, where particles are accelerated or heated via magnetic reconnection and/or magnetohydrodynamic (MHD) instabilities. This work shows in great detail how the final spectrum is generated by a magnetized jet as it expands (see the figure). It clearly demonstrates that if electrons are accelerated into a relativistic power-law energy distribution, then synchrotron emission is the dominant radiation mechanism (left panel). Alternatively, if electrons are heated into a warm plasma (all particles having the same average energy), then the prompt GRB spectrum forms via Compton scattering of thermal photons (right panel). The shown spectra are obtained from a specially designed numerical code that accurately accounts for all relevant high-energy processes, including production and annihilation of electron-positron pairs, in a relativistically expanding jet.
The way the low- and high-energy (below and above the spectral peak) parts of the spectrum are generated has important implications for energy-dependent linear polarization. In the left panel, synchrotron emission can yield strong linear polarization, depending on the magnetic field geometry. In the right panel, however, multiple Compton scatterings wash out any polarization and only produce a negligible global polarization in a uniform (with no local angular structure) jet. Using these results, more sensitive spectro-polarimetric observations from detectors onboard upcoming space missions will help to pin down the exact prompt GRB radiation mechanism.
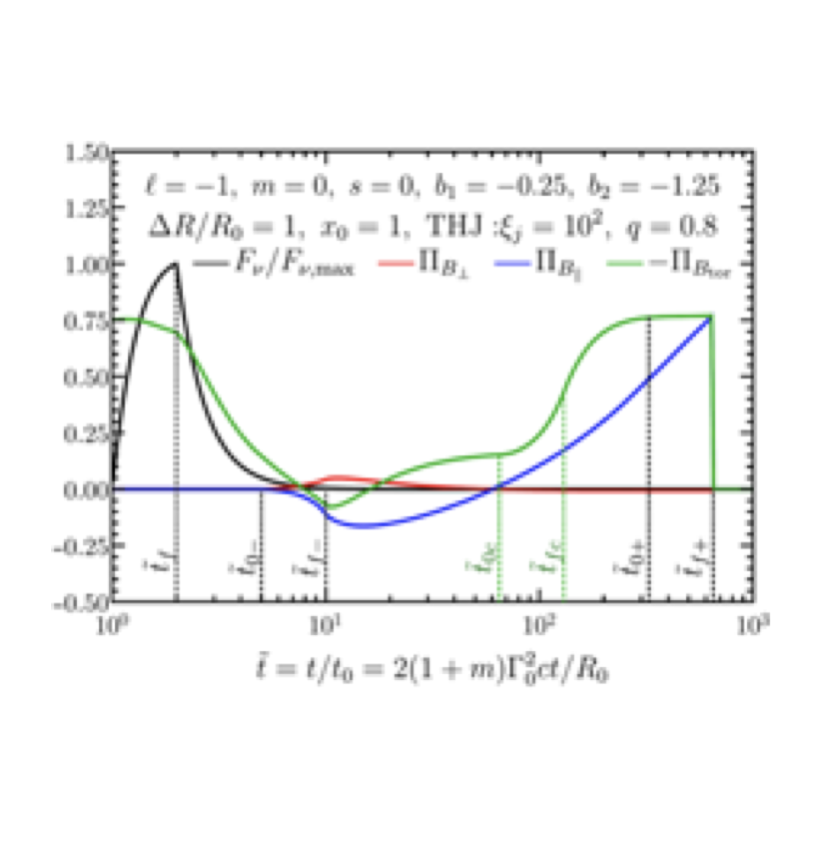
GRB Polarization: A Unique Probe of GRB Physics
Gamma-ray bursts (GRBs) are the most powerful explosions in the Universe. They are powered by ultra-relativistic jets (highly collimated outflows moving at ~99.995% of the speed of light). The huge isotropic-equivalent gamma-ray luminosities of Lɣ,iso~1051 - 1054 erg/s of their brightest prompt emission phase places GRBs as the most (electromagnetically) luminous transient events in nature. This makes them detectable out to the far reaches of the Universe, from down to barely a billion years after the Big Bang. The exact radiation mechanism that produces this prompt emission is still a mystery. The two most popular candidates are synchrotron emission from relativistic electrons with a power-law energy distribution and Compton scattering of thermal radiation by warm electrons. Both mechanisms can explain the majority of the observed prompt GRB spectra equally well, making it hard to distinguish between them. However, they can lead to very different linear polarization, depending on the magnetic field geometry (for synchrotron emission) and the jet’s angular structure (for both). Therefore, high-sensitivity linear polarization measurements can help distinguish between them and reveal the dominant radiation mechanism. Moreover, for synchrotron emission they can also teach us about the magnetic field structure in the emission region.
In this work, Dr. Ramandeep Gill (ARCO), Dr. Merlin Kole (University of Geneva) and Prof. Jonathan Granot (ARCO) provide a comprehensive review of the predictions for prompt GRB linear polarization from theoretical models and the current status of polarization measurements. The work gives a concise overview of the fundamental questions in GRB physics, namely what are the jet composition and dynamics, how and where is the energy dissipated, and what are the different candidate radiation mechanisms capable of producing the non-thermal prompt GRB spectrum. It presents relevant formulae and polarization predictions (both time-integrated and time-resolved) for different radiation mechanisms, magnetic field configurations (relevant for synchrotron emission), jet angular structures and dynamics. On the observational front, this work presents the basics of gamma-ray polarimetry and chronicles the GRB polarization measurements made by different detectors over the years. The figure shows example lightcurve (black) and time-resolved polarization (Π) curves for synchrotron emission from different magnetic field configurations in one realization of a uniform (lacking angular structure) jet seen at an angle from the symmetry axis of the jet (for a single pulse in the GRB prompt emission lightcurve).
The detailed polarization predictions presented in this work will enable observers to compare high-sensitivity measurements from upcoming gamma-ray polarimeters with theoretical models and constrain fundamental properties of the GRB relativistic jet and prompt emission mechanism.
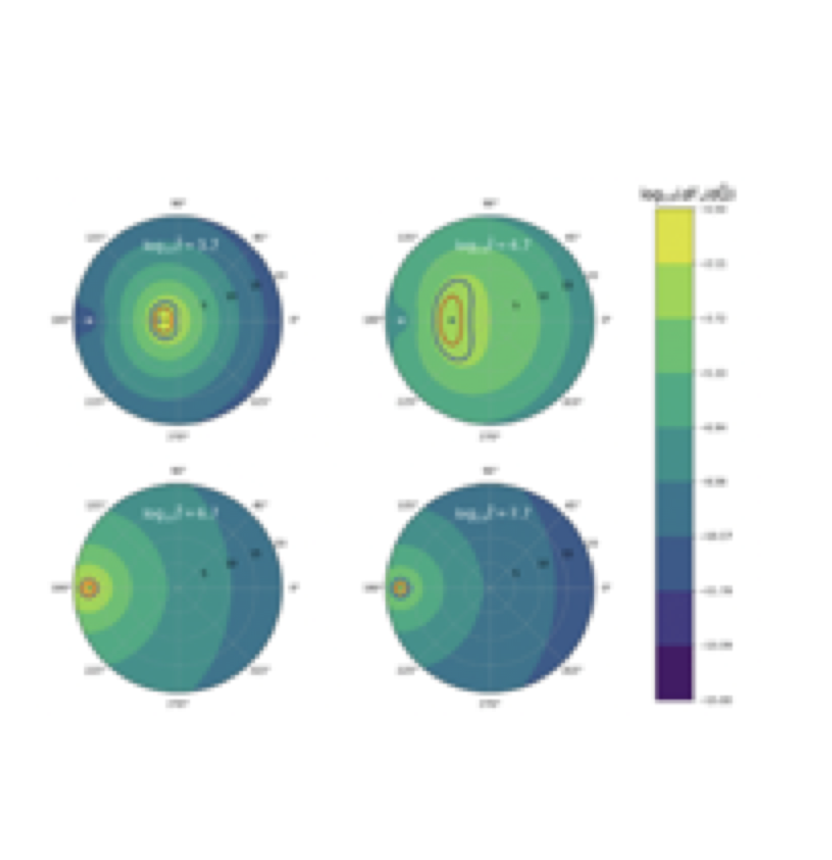
Gamma ray burst afterglows from neutron star merger jets
One of the key results of Einstein’s general theory of relativity was the prediction of gravitational waves (GWs) – `ripples’ in space-time that travel at the speed of light and carry gravitational energy. Such waves are abundantly created when compact massive objects are accelerated. Mergers of very dense objects, such as black holes and neutron stars are an especially prolific source of GWs. Almost 100 years after their prediction by Einstein, they were directly measured for the first time in 2015, coming from a pair of merging black holes, by the LIGO interferometer. This groundbreaking discovery was awarded the Nobel prize in physics in 2017. It was on the 17th of August 2017 that GW detectors observed another type of cataclysmic event: the merger of two neutron stars.
Such mergers are particularly interesting, as they are linked to some of the brightest electromagnetic transients in the Universe - short gamma-ray bursts (GRBs). In short GRBs jets traveling at very close to the speed of light create high-intensity radiation (mainly in gamma rays at first and later across the electromagnetic spectrum, in the stage known as the GRB afterglow). The beaming of light emitted by objects moving at near-light speeds, combined with the jetted geometry of the emitting material, implies that we typically detect such bursts `face on’, where our line of sight lies within the jet’s core. GRBs are so bright that they are typically seen from distances of billions of light-years from Earth. Although GRBs have been observed for over 50 years, some of the most basic questions about their nature remain unsolved, including the structure and composition of their jets and the physical process powering different phases of their emission.
[caption id="attachment_1166" align="alignright" width="647"] Figure 1 Flux per unit area of the sky observed from an afterglow of a structured jet viewed off-axis at different times. The observer is initially dominated by the low energy material that is close to their line of sight (top left). Over time, as the jet slows down, and relativistic beaming less prominent, the emission becomes dominated by the energetic core (denoted by a white cross).[/caption]
The event observed in 2017, dubbed GW170817, based on its detection date, lead astronomers to point a variety of electromagnetic telescopes to the same location on the sky. Indeed, a short GRB was detected, confirming the link between these two phenomena. However, this GRB was different than any of the previously seen bursts. In particular, the initial gamma-ray emission was much less luminous and the afterglow `light-curve’ (the temporal evolution of the recorded electromagnetic signal) evolved in a distinct way, initially rising gradually for over 150 days and then decaying rapidly. The reason for this difference is that the detection trigger by the GWs enabled astronomers for the first time, to view a GRB jet, quite literally, from a different point of view: from a line of sight far away from the jet axis (off-axis; see figure 1) and coming from a distance much closer to Earth. This enabled us, in turn, to learn in unprecedented detail about the lateral structure of the underlying GRB jet and to better understand how such jets propagate through their environments and develop this structure.
The future of binary mergers jointly detected in GWs and as GRBs holds much promise. An investigation, that Dr. Beniamini, Prof. Granot and Dr. Ramandeep Gill (all members of ARCO) carried in “Afterglow light curves from misaligned structured jets” derives in detail the lightcurves from the accompanying GRB afterglows from jets with different lateral structures, observed from arbitrary viewpoints. Using analytical tools, the study reveals qualitatively different types of light curves that can be viewed in future off-axis GRBs, with either single or double peaks (Figure 2), depending on the jet structure and the viewing angle. It shows that focusing on the shape of the lightcurves from such jets rather than absolute times or fluxes, enables to deduce intrinsic properties of the jet and its angle to the observer, independently of many of the degenerate and therefore unconstrained physical parameters that affect the afterglow properties of `standard’ GRBs. Such robust parameter inferences will provide a crucial stepping stool for numerical investigations, attempting to deduce the underlying physical properties of individually observed GRBs. At the same time, our study can help guide future observational transient survey campaigns, attempting to search for `orphan afterglows’ – GRBs in which the early burst of gamma rays is missed (as the jet points away from Earth) but are detected later in shorter wavelengths (e.g. X-ray, optical or radio) during the afterglow phase when the jet decelerates and its radiation beam widens and reaches our line of sight.
Figure 2 Different types of light-curves that can be viewed by observers misaligned with the jet core. Focusing on the temporal slopes, as well as the ratio of the characteristic times and frequencies provides robust information on the jet properties and observer’s viewing angle, independently of the many other unconstrained physical parameters.
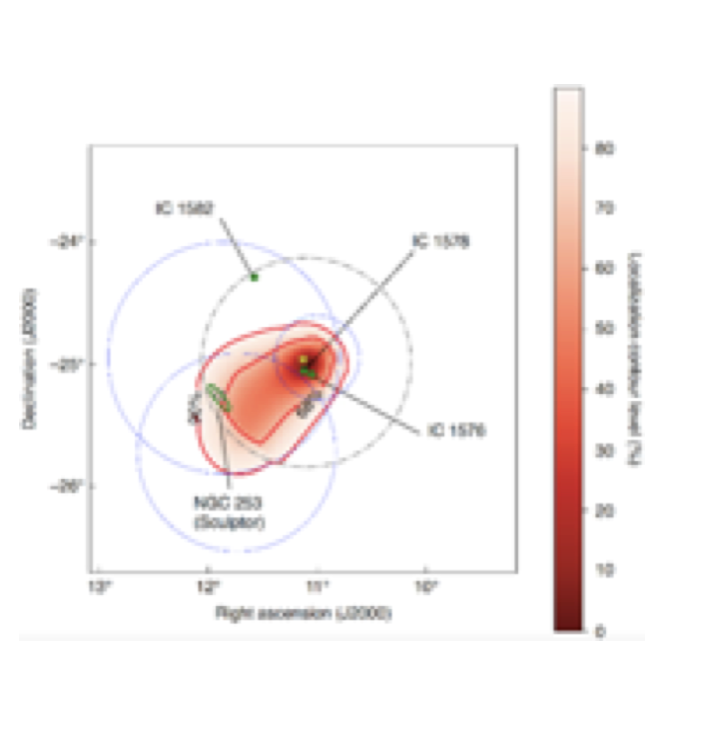
High-energy emission from a magnetar giant flare in the Sculptor galaxy
Magnetars are young neutron stars with the strongest magnetic fields in the Universe (surface fields of 1013–1015 G). They probe some of the most extreme physical conditions in nature, such as strong field gravity, huge (nuclear) densities and extreme energy densities. The evolution and decay of their ultra-strong magnetic field powers a variety of X-ray bursting activity, ranging from relatively frequent short bursts to very rare and much more energetic giant flares (GFs), which can dissipate a good part of their magnetospheric energy and eject plasma at relativistic speeds.
Magnetar GFs consist of a short-duration (0.1–0.5 s) bright initial spike followed by a longer (300–400 s) and much dimmer pulsating tail, strongly modulated at the magnetar’s known spin period (of typically a few to several seconds). So far three GFs were clearly recorded – two Galactic and one from the Large Magellanic Cloud (LMC) – a small satellite galaxy of our Milky Way. The most recent and energetic of these events, detected on Dec. 27, 2004, from the Galactic magnetar SGR 1806–20, produced measureable changes in Earth’s upper atmosphere despite erupting from a magnetar located about 40,000 light-years away. The two Galactic GFs showed longer lasting (days to many months) radio emission, which provided good evidence for the ejection of plasma at mildly relativistic speeds (about 50%–70% of the speed of light, close to the escape velocity from the neutron star) with a kinetic energy comparable to the energy radiated in the GF’s initial spike.
This work reports the first high-energy (with photon energies of the order of giga-electronvolt – GeV) detection of emission from a magnetar GF. This event was from the nearby (3.5 megaparsecs – Mpc) Sculptor galaxy, which is much further away than the Galactic GFs (10-15 kiloparsecs – kpc) or the GF from the LMC (50 kpc), and therefore only its initial spike was bright enough to be detected while its pulsating tails was too dim. The Large Area Telescope (LAT) on board the Fermi Gamma-ray Space Telescope detected GeV γ rays from 19 s until 284 s after the initial detection of a signal in the megaelectronvolt (MeV) band (which lasted 0.14 s, corresponding to the GF’s initial spike). Our analysis shows that these γ rays are spatially associated with the Sculptor galaxy and are unlikely to originate from a cosmological γ-ray burst. Thus, we infer that the γ rays originated with the magnetar GF in Sculptor.
A magnetar produces a steady magnetized wind powered by the very gradual slowing down of its rotation. As the magnetar moves through space, this wind plows into, slows, and diverts interstellar gas. The gas piles up, becomes heated and compressed into a thin curved shell, and forms a type of oblique shock wave called a bow shock.
In the model proposed by the LAT team, with leading roles by Prof. Jonathan Granot and Dr. Ramandeep Gill of ARCO at the Open University of Israel, the GF’s MeV initial spike travels outward
at the speed of light, followed by the cloud of ejected matter consisting of protons, electrons and positrons, which is moving nearly as fast (at about 99.995% the speed of light). After several days, they both reach the bow shock. The MeV γ rays pass through. Then, several seconds later, the cloud of particles – now expanded into a vast thin shell – collides with gas at the bow shock shell. This collision between the two shells drives shock into both of them that accelerates particles, which produce the highest-energy GeV γ rays several seconds to minutes after the main burst.
This new and exciting event shows both similarities and differences relative to the magnetar GFs in our Milky way galaxy. On the one hand, in all of these events the energy radiated in γ rays and the kinetic energy of the ejected matter are comparable. On the other hand, in this new event the ejected matter is much faster, at about 99.995% of the speed of light, compared to about 50%–70% of the speed of light in the two GFs in the Milky way. This much faster velocity implies, in turn, a much smaller amount of protons in the ejected matter, which demonstrates a much wider diversity between different magnetar GFs than was known so far.
This work was published in Nature Astronomy
See also a short illustrative video.
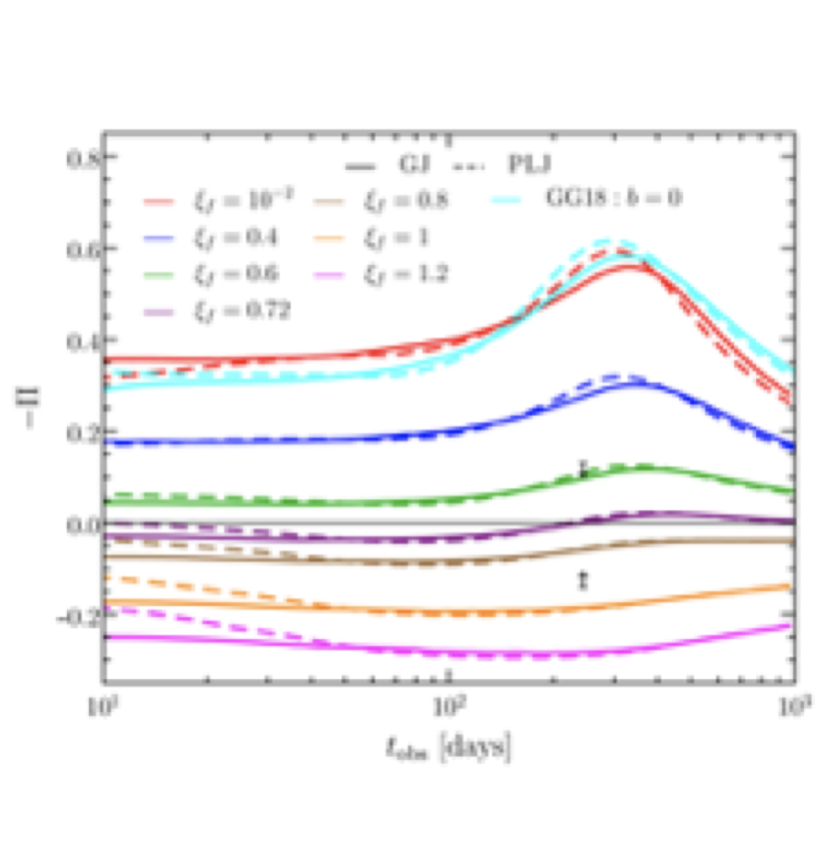
Constraining the magnetic field structure in collisionless relativistic shocks with a radio afterglow polarization upper limit in GW 170817
Shocks (a.k.a blast waves) are a ubiquitous phenomenon in the Universe. In many astrophysical objects, emission all the way from radio through X-rays to very high energy gamma-rays is powered by collisionless shocks (which are mediated by collective electromagnetic interactions rather than by particle collisions). These include relativistic (traveling at very close to the speed of light) blast waves powered by stellar-mass black holes in gamma-ray bursts (GRBs) and supermassive black holes in active galactic nuclei, or non-relativistic blast waves in supernovae.
It is well established that the broad-band (radio to gamma-rays) afterglow emission in GRBs is produced by relativistic electrons that are accelerated at collisionless shock fronts. In particular, these electrons emit synchrotron radiation as they gyrate in the shock-generated magnetic fields behind the shock front. Several theoretical works and numerical (particle in cell - PIC) simulations are devoted to understanding the structure of this magnetic field, which is posited to be tangled and anisotropic - constrained entirely to the plane of the shock - perpendicular to the shocked fluid’s local velocity. Since synchrotron emission is generally partially linearly polarized, this has important implications for the measured degree of polarization (Π). GRB afterglows show polarization of up to a few percent, which already suggests that the shock-generated field is closer to isotropic (a fully isotropic field gives Π = 0) than anisotropic. Better understanding of the magnetic field structure in GRBs, the majority of which have been observed at distances of billions of light-years, is limited by our incomplete knowledge of the angular structure of the relativistic jet and our viewing angle relative to its symmetry axis, which also affects the degree of polarization Π, thereby creating a degeneracy.
A golden opportunity was provided by the afterglow of GW170817 / GRB 170817A that originated in the aftermath of a merger between two neutron stars only 130 million lightyears away from us. Dr. Ramandeep Gill and Prof. Jonathan Granot (both from ARCO) used the afterglow from this source to study the properties of the relativistic jet, including its angular structure, in several joint works. In the present work, they used their knowledge of the jet’s angular structure (either a gaussian jet (GJ) or power-law jet (PLJ) and our viewing angle (about 25 to 30 degrees or 5 to 6 times the jet’s core angle), to isolate the effects of the shock-generated magnetic field structure on the afterglow linear polarization. The figure shows several polarization curves marked by ξf, the parameter characterizing the anisotropy of the magnetic field (becoming more anisotropic away from unity) just behind the shock front. The two arrows show the upper limit on the afterglow polarization obtained from radio observations. This work improved the constraints on the magnetic field anisotropy (0.57 < ξf < 0.89) and clearly showed that the magnetic field just behind the shock front is only mildly anisotropic, contrary to several earlier works.
The implications of this study are of fundamental importance for collisionless shock physics. In particular, it suggests that the magnetic field may be produced not only by small-scale plasma instabilities but is instead also strongly affected by macroscopic turbulence in the region behind the shock front, which generally yields more isotropic magnetic fields.
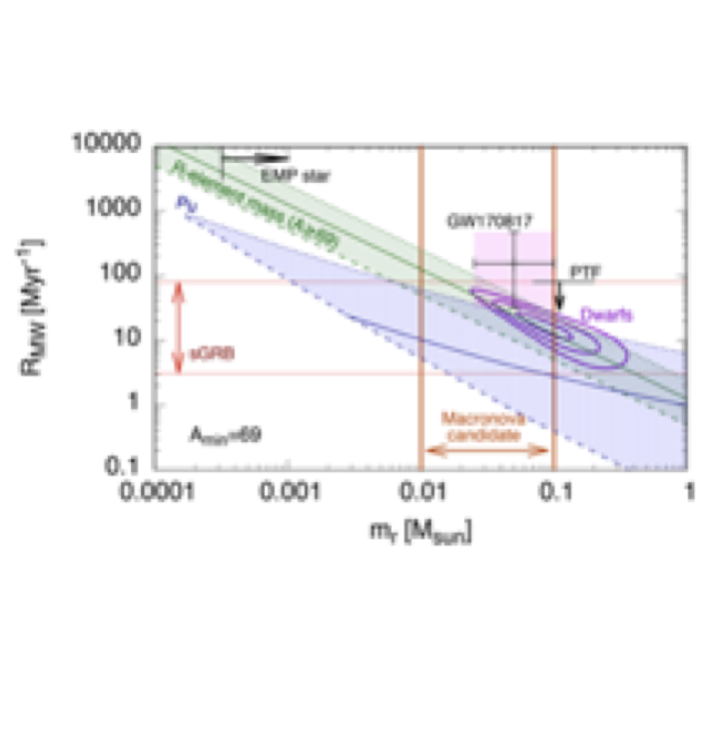
The rate of r-process production in the Universe
[caption id="attachment_759" align="alignright" width="425"] Figure 1 Colored regions denote different constraints on the rate and r-process mass produced per event (from the review by Hotokezka, Beniamini & Piran, 2018). The constraint from UFDs is shown by purple contours and that from GW 170817 by a pink square.[/caption]
Since the dawn of humankind, people have been fascinated by the origin of gold. It appears prominently in mythologies all over the world: from the tears of the sun god Inti in the Incan culture to the cursed golden touch of king Midas in Greek mythology. This obsession resulted in a pursuit to try and artificially create gold that despite never being successful, was common enough to get its own term – alchemy. Modern physics provided us with the atomic theory, which teaches us that the distinction between different elements lies in the number of protons in their nucleus. Different types of atoms may indeed be transformed from one type to the next (as imagined by ancient followers of alchemy, although in a completely different manner), through nuclear processes such as fusion and fission.
In the astrophysical context, the formation of different elements, are dominated by different processes. For example, while most of the H and He in the Universe are relics of the big bang, slightly heavier elements are formed mainly through cosmic spallation or through nuclear fusion in the hearts of stars. Elements heavier than iron require an external source of energy to form and can therefore only occur in environments with very large densities and temperatures. About half of these elements (including gold) are dominated by the so-called rapid (or for short r) process, which requires extreme physical conditions in order to take place.
Since the fifties it has been thought that this process may take place in supernovae – the explosions that mark the end of massive stars’ lives. However, over the years, it gradually became clear that the required conditions for r-process are not readily achieved in standard supernovae. Furthermore, in the last several years, there has been growing evidence in an altogether rarer and much more exotic (but also potentially much more prolific) channel – mergers of binary neutron star systems (see figure 1).
The r-process element abundances in stars in our own Galaxy is well measured. Nonetheless it does not immediately reveal the rate of the astrophysical process behind their formation. Broadly speaking, the process may either be very common but with each event creating a meager amount of r-process
material or else the events may be rare but individually prolific. In a 2020 study, Dr. Beniamini and collaborator have shown that data from Milky Way stars can be used to constrain the rate of r-process events, as well as constrain the speed of their mixing in the interstellar medium. This is done
through several techniques. First, they consider the scatter of r-process abundance (of stable r-process elements) between stars with similar iron abundances (which were therefore created in most cases, around a similar time). A greater rate of r-process events and / or speed of their mixing, leads to a smaller scatter, and vice versa. Secondly, if the speed of mixing were slow and / or the rate of enrichment high, then a fraction of the observed stars should have been formed in areas that are still greatly enriched from the latest enrichment event. This would lead to stars with huge levels of r-process enrichment which are not observed. This condition can therefore rule out combinations of sufficiently slow mixing and rapid enrichment rate. A third constraint comes from studying isotope abundance ratios as compared to their formation ratios. A greater ratio indicates a lower r-process enrichment rate and / or mixing speed. Combining all these considerations together allows to put strong constraints on the rate of r-process enrichment and the speed of mixing of those elements in the interstellar medium, which are once more consistent with neutron star mergers. Studies such as this bring us closer to deciphering the origin of gold and other r-process in the Universe and critically test our astrophysical theories.
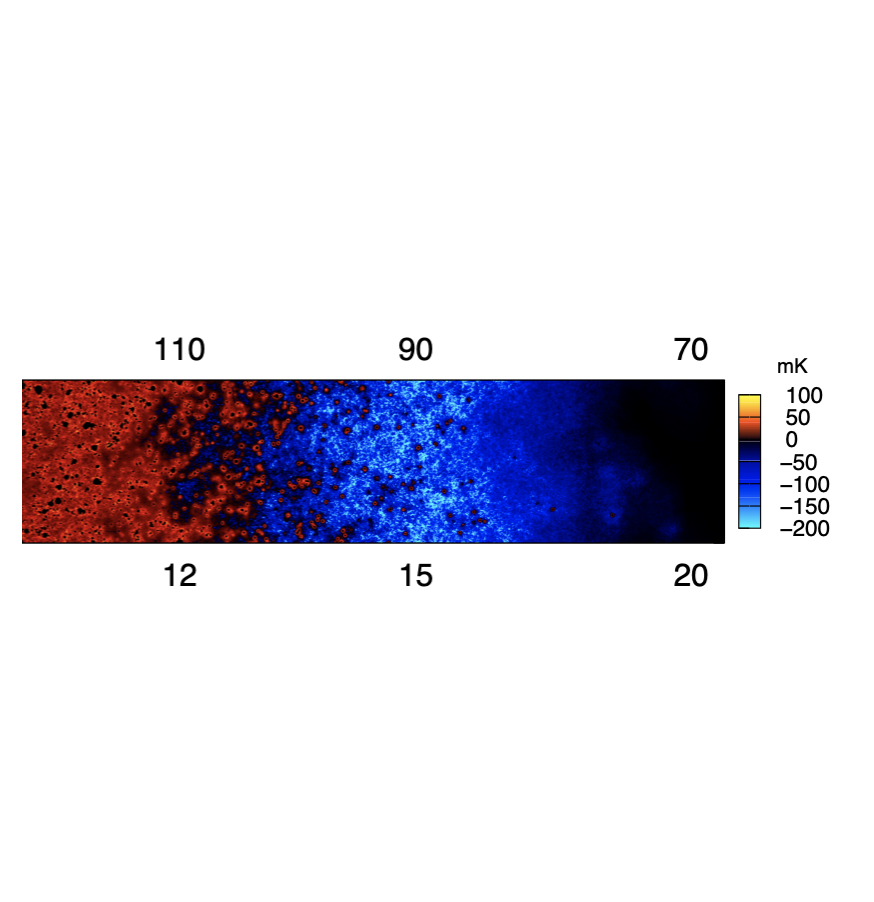
Exploring the epoch of hydrogen reionization using FRBs
Immediately after the big bang, the Universe was filled with extremely dense and hot ionized gas. As the universe expanded and cooled, electrons, protons and neutrons became less energetic and for the first time were able to combine to form neutral atoms. This era which took place only 380,000 years after the big bang and is known as the era of recombination, also marked the first time in the Universe’s history at which light was able to travel freely through space. However, at this point in the Universe’s history, there was little light to speak of. Only a few hundred million years later, as dense clouds of gas collapsed into the first stars, light was finally being created in abundance. The higher frequency UV light created by these first stars split hydrogen atoms back into electrons and protons, in a process known as ionization. As the electrons and protons were already separated from each other earlier in the evolution of the Universe (before the recombination era), this stage of the Universe is known in cosmology as “the era of reionization”. Reionization presents one of the few means by which we can (indirectly) study the conditions in the Universe very early on and the properties of the earliest stars that lived in it. However, the details of when exactly reionization occurred and how it proceeded over time and space thereafter, are still poorly constrained by data.
[caption id="attachment_1175" align="aligncenter" width="800"] Figure 1 Simulation of cosmic reionization showing temperature variations as a function of cosmic redshift (larger values correspond to earlier cosmic times) for a given ‘slab’ of the Universe. Image courtesy of Raghunath Ghara and Saleem Zaroubi.[/caption]
Fast Radio Bursts (FRBs) are brief (typically lasting a few milliseconds), very bright radio pulses arriving at Earth from billions of light-years away. The FRB field is relatively new (with the first detection taking place in 2007) and many properties of FRBs are still a topic of active investigation. Nonetheless, their connection to highly magnetized neutron stars – ‘magnetars’ was vividly established in April of 2020, when a known magnetar source in our own Galaxy, was observed to produce such a burst. Magnetars are known to be a common by-product of the collapse of massive stars (see work by Dr. Beniamini and collaborators), which in turn are thought to be a major contributor to the Universe’s reionization as mentioned above. Indeed, the conditions taking place in the early Universe are likely to make the extremely strong magnetic fields needed to power magnetars even easier to attain. Furthermore, some gamma-ray bursts, are known to take place during this era, and the creation of their jets almost definitely requires enormous magnetic fields. The existence of magnetars in the Early Universe, combined with the great abundance of FRBs (which are created at a rate of about 10,000 per day over the entire sky, several thousands of times more common than, for example, gamma ray bursts), and the relative ease of detecting them from large distances, make FRBs excellent probes of cosmology. On top of this, for each FRB, astronomers get a measurement of the “dispersion measure”, a quantity which reflects the number of ionized electrons in a column between the FRB source and us, and which for remote FRBs, can illuminate the properties of the intervening intergalactic medium, which is not readily observed by other means.
[caption id="attachment_1176" align="alignleft" width="213"]
Figure 2 Top: Different possible histories for the fractions of ionized electrons as a function of cosmic 'redshift' (higher redshift corresponding to an earlier stage of the Universe). Bottom: The dispersion measures associated with these ionized electron fraction histories.[/caption]
In a work from 2021, Dr. Beniamini and collaborators have shown that the distribution of “dispersion measures” of FRBs from the early Universe holds great potential towards exploring the era of reionization. One aspect they explore, makes use of the fact that due to the reionization of the Universe taking place at a finite time, there is a maximum limit (which depends on the reionization history) to the column of ionized electrons that can lie between us and an FRB source (if the FRB is very distant, then the FRB signal was created before the Universe was fully reionized and the burst travelled a portion of its journey towards us through mostly neutral material). The research outlines three different methods, using either FRBs with known or unknown host galaxies which can be used to measure the Universe’s reionization history. FRBs have the potential to become the most accurate source of information about the era of reionization in a matter of several years.